Maximise Interface Stability in All-Solid-State Lithium Batteries
Technical Analysis | 08-10-2024 | By Liam Critchley
All-solid-state batteries (ASSBs) are seen as one of the key battery architectures that could address the energy density challenges of Li-ion batteries. In ASSBs, the liquid or gel electrolyte that is found in Li-ion batteries is replaced with a solid material. There are many different types of solid electrolytes that can be used, including ceramics, polymers, resins and glass composites.
While Li-ion batteries are currently the commercial gold standard due to possessing a good energy density and being relatively stable, the liquid nature of the electrolyte does mean that some Li-ion batteries can leak, form dendrites and undergo thermal runway—all of which pose a safety risk and reduce the usable lifetime of the battery. Solid electrolyte materials are not flammable and can help reduce the chance of batteries catching fire, but there is also the potential to increase the energy density and lifespan of batteries compared to the status quo.
Key Things to Know
- ASSBs use solid electrolytes instead of liquid or gel, which can improve safety and energy density.
- Solid electrolytes reduce the risk of fire by preventing dendrite formation and thermal runaway.
- There are multiple solid electrolyte types, including ceramics, polymers, and glass composites, each with distinct advantages.
- Compared to traditional Li-ion batteries, ASSBs promise longer lifespan and higher energy storage potential, addressing critical industry challenges.
Poor Interfacial Stability The Challenge for ASSLBs
There are many different all-solid-state lithium batteries (ASSLBs) configurations, but those based on garnet-type solid-state electrolytes are considered to be among the safest alternatives to conventional Li-ion batteries. This is typically due to their inherent non-flammability. However, even though many ASSLBs are considered to be potentially safer than today’s commercial Li-ion systems, they typically suffer from poor interfacial stability between the positive electrode and the solid electrolyte.
This poor interfacial stability arises due to the need for a trade-off between chemical stability and wettability. This often requires an energy-intensive and high-temperature sintering process to achieve sufficient interfacial contact between the electrode and electrolyte. The interface between the electrode and the electrolyte plays an important role in ASSLBs, but trading off the level of contact for chemical stability leads to a poor cycling performance—hence, it needs to be addressed.
Previous Studies Make Progress but No Breakthrough
The need for addressing interfacial stability has led to many studies in the field. Previous studies have suggested that traditional positive electrode materials can react with garnet-type electrolytes, causing a chemically stable temperature lower than the wetting temperature—which leads to unstable and poor cycling performance.
Low melting point solder has been attempted to improve the contact, but this led to a reduced capacity due to there being a lower amount of active electrolyte material in contact with the electrode. Ultrafast high-temperature sintering (UHS) with Joule heating has helped to prevent cross-diffusion at the interface but a trade-off was still apparent in this approach. Based on some of the latest studies to date, it’s thought that high entropy materials might offer an answer to the interface stability without the need for some kind of property trade-off.
New Study Combines Thermodynamics and Kinetics to Improve Interface Stability
High entropy materials composed of different components have gathered some attention for ASSLBs because the synergistic effects of multiple material elements can be exploited. High entropy materials are known to maximise the configurational entropy and form highly stable structures while also exhibiting a high thermal stability and low sintering temperature.
As a positive electrode material, high entropy cationic disordered rock salt has shown a lot of promise due to its excellent lithium storage properties. Due to the unique entropy effects seen in high-entropy materials, they have the potential to achieve a much better balance between chemically stable temperature (i.e. the chemical stability) and a low wetting (the wettability aspect).
The Balance Between Chemical Stability and Wettability
The challenge of balancing chemical stability and wettability at the interface is particularly significant in high-performance solid-state batteries. Recent advances in using high entropy materials, such as disordered rock salts, offer an innovative approach to overcoming these issues. High entropy materials reduce the temperature gap between the stability and wettability thresholds, leading to more efficient lithium-ion transfer at the electrode-electrolyte interface, ultimately enhancing the overall cycling performance of the battery.
In a recent study, researchers have been able to achieve a thermodynamic compatibility and good interfacial content between high entropy cationic disordered rock salt positive electrodes and an LLZTO solid electrolyte using UHS. The approach combined fast reaction kinetics and thermodynamics (entropy stabilisation) to build a highly stable electrode-electrolyte interface. This is due to a favourable balance of chemical stability and wettability being achieved.
UHS not only aids in preventing element cross-diffusion but also ensures a smooth interface between the electrode and electrolyte. This precise control of the reaction environment significantly enhances interface conductivity, a critical factor in reducing resistance and maintaining battery efficiency over extended cycles. The effectiveness of UHS in managing thermal loads, while maintaining low resistance at the interface, marks a substantial step forward in addressing the interfacial instability commonly seen in conventional ASSLBs.
The Benefits of UHS in Preventing Element Cross-Diffusion
The UHS approach enables the precursor electrode materials to undergo a rapid in-situ phase formation reaction which produced a conformal interface on the surface of the electrolyte. The heating and cooling process used throughout the reaction also helped to prevent any elemental diffusion across the interface. The short annealing time of 3 seconds enabled the interface to have a chemically stable temperature of 1100 °C.
The construction of a stable interface between high-entropy disordered rock salts (HE-DRX) and the garnet-type LLZTO solid electrolyte is key to improving the performance of all-solid-state lithium batteries. The ultrafast high-temperature sintering (UHS) process plays a critical role in this achievement by allowing the HE-DRX positive electrode to form rapidly on the surface of LLZTO. This process minimises the risk of side reactions and ensures that the interface remains conformal, thereby enhancing the overall stability and efficiency of the battery. The schematic below illustrates the construction of this stable interface using UHS technology.
Such rapid annealing prevents the formation of secondary phases at the interface, which could otherwise degrade battery performance. By maintaining an even distribution of elements across the interface, UHS enables the full utilisation of the electrode’s active material. This process minimises the occurrence of unwanted reactions that typically arise when operating at higher temperatures, further contributing to the longevity and stability of ASSLBs.
High Capacity Retention in ASSLBs Using High Entropy Materials
Moreover, this high capacity retention highlights the potential of high entropy materials to sustain prolonged battery life without significant degradation. As the automotive and energy storage sectors seek more reliable power sources, maintaining over 95% capacity retention after 100 cycles marks a breakthrough in solid-state battery technology. This retention rate is especially promising for applications that demand both high power and longevity, including electric vehicles and grid energy storage systems.
The electrode-electrolyte interface was found to be very stable, with no side reactions occurring. This approach reduced the interface resistance to 31.6 Ωcm2 at 25 °C, which is a reduction of 700 times compared to other interfaces that use LLZTO with more traditional electrode materials (such as LiCoO2). The conformal and tight electrode on the electrolyte surface also avoids transition metal migration—something which is common with liquid electrolytes.
Enhancing Battery Efficiency and Reducing Resistance
By lowering the interface resistance to such a significant degree, the UHS method ensures that energy losses during charge and discharge cycles are minimised. This development is critical in advancing the feasibility of solid-state batteries for applications requiring high energy density and fast charging capabilities. The reduced resistance also contributes to maintaining the structural integrity of the battery, particularly during repeated cycling, where heat build-up can compromise both performance and safety.
When put together as a complete ASSLB, the electrode-electrolyte interface engineered in this study produced a battery with an average specific capacity of 239.7 ± 2 mAh/g at 25 mA/g and a capacity retention of 95% after 100 cycles at 150 °C—which is much higher than liquid electrolyte batteries that only retain around 76% capacity after 20 cycles at 25°C. Using both thermodynamic and kinetic principles could open new doors for tackling interface issues in a range of ASSLB architectures in the near future.
Conclusion
All-solid-state batteries (ASSLBs) represent a key advancement in addressing the limitations of traditional lithium-ion batteries, particularly in terms of energy density and safety. The development of high entropy materials, such as disordered rock salts, in combination with advanced techniques like ultrafast high-temperature sintering (UHS), offers significant improvements in the stability and efficiency of the electrode-electrolyte interface. By balancing chemical stability and wettability, these innovations are pushing the boundaries of what is possible with solid-state battery technology.
The ability to lower interface resistance, enhance battery efficiency, and ensure long-term capacity retention demonstrates the vast potential of these materials in high-demand applications, including electric vehicles and grid energy storage. As research continues to evolve, the combination of thermodynamic and kinetic principles could pave the way for even more breakthroughs in ASSLBs, bringing us closer to safer, more efficient, and longer-lasting energy solutions.
Reference:
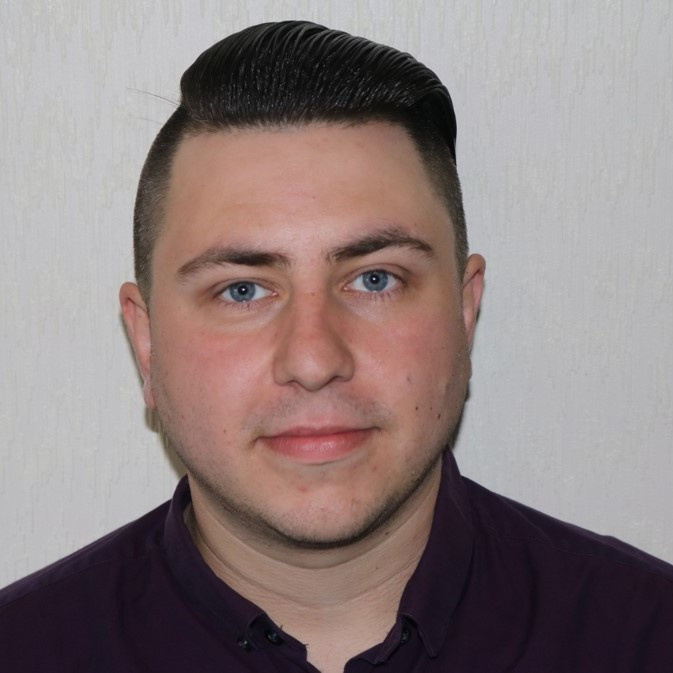