Whitepaper: A Guide to Direct and Coherent Detection in LIDAR Systems
26-03-2024 | By Jack Pollard
LIDAR technology is reshaping the future of automotive safety and autonomous navigation. In this comprehensive whitepaper, Harvey Weinberg of MicroTech Ventures and Pier-Olivier Hamel from indie Semiconductor dissect the nuances between direct and coherent detection methods in LIDAR systems, providing an essential guide for industry professionals seeking to navigate this complex landscape.
Introduction
As LIDAR has become a hot topic in the sensor world, mostly thanks to efforts in the ADAS and autonomous driving sector, a debate has emerged as to whether direct-detection (or time-of-flight) or coherent (Frequency Modulated Continuous Wave, for example) photon detection is best. In truth, “best” depends very much on the application. LIDAR is used in a wide variety of applications from traffic management, to driver assistance and autonomous driving, to ground mapping, to meteorological applications. It should be no surprise that the importance of different LIDAR performance metrics – maximum range, accuracy, interference immunity, cost, etc. – vary from application to application. Even within the same application, certain system choices may skew the importance of one parameter or another. This paper aims to discuss the different characteristics of direct and coherent detection in order to educate those interested in LIDAR and allow them to make informed system choices.
Historical Background
LIDAR was first conceptualised in the 1930’s – around the same time as radar was developed. However, it wasn’t until the early 1960’s, when the first lasers were developed, that LIDAR became a reality. Coherent Frequency Modulated Continuous Wave (FMCW) radar had been developed in the mid-1930’s and shortly thereafter researchers set to work to bring the benefits of coherent detection to light-based ranging. During the 1960s, multiple researchers were demonstrating early FMCW LiDAR systems. Since then, LIDAR has found use in dozens of applications, each one with its unique list of challenges that developers had to overcome. The rise of optical telecommunications gave another boost to LIDAR from developments in advanced lasers and improved modulation techniques funded by the huge number of research dollars poured into optical telecommunications. Just as with radar, early optical telecommunications relied on pulsed, direct detection techniques. By 2008, coherent detection started to take over. Today the industry is fortunate to have the benefit of over half a century of development in hardware and signal processing advances that were developed for radar and optical communications to draw on in bringing the “next” LIDAR system to life.
Photon Detection
At its most basic, a LIDAR system emits photons and calculates how long those photons took to reach a target and return. While there are many aspects of a LIDAR system to consider (what wavelength to use, scanning method, how to deal with interference, etc.), the choice of how we detect those returning photons drives almost every other system choice. There are, essentially, two methods of photon detection:
Direct detection: A laser pulse is fired which starts a timer. The timer is stopped when the echo of the laser pulse is received. We do not consider the phase of the photons, simply their existence (amplitude) and return timing. As the speed of light is known and invariant, we calculate the distance to the target as Δt C 2 where Δt is the time between the start of photon transmission and the leading edge of photon reception (as shown in Figure 1).
Coherent detection: A modulated laser is on for a longer period of time and the return signal is optically mixed with a sample of the transmitted photodetection (called the local oscillator) before photodiode detection. This optical mixing results in the receive signal being amplified by the local oscillator. By using a sample of the transmit signal we are assured that the phase relationship between transmit and receive channels is preserved (or coherent). As with direct detection, distance is calculated by measuring the time between photon transmission and reception. But in the case of coherent detection, modulation is applied to the continuously (or quasi-continuously) transmitted signal. As the laser is transmitting continuously the echo timing is determined by appropriate demodulation, which requires more signal processing than direct detection. With coherent detection, we can directly measure velocity instantaneously (not by measuring target movement over multiple frames as one would do with direct detection) by detecting the frequency shift of the returned signal caused by Doppler.
We will begin by describing direct detection in detail as it is conceptually simpler.
Direct Detection
Direct detection systems employ a pulsed laser to emit short bursts of light (a few nanoseconds). The LIDAR sensor measures the time needed to receive the reflected light pulse. By analysing the time it takes for the light to travel to the target and back, it calculates the distance to objects in the environment.
Direct detection is suitable if only modest performance is needed, such as a range under 50 meters. There is no need for a tunable, single-mode laser since the primary requirement is merely a source of numerous photons within a short timeframe. Consequently, the laser driver circuit is simplified as there is no necessity to modulate the laser; instead, the modulator's task is to rapidly inject a significant amount of current into the laser. Additionally, the precision requirements for optics are reduced, as concerns about wavefront distortion are minimal.
Mathematically, the return power in a direct detection-based system can be expressed as follows:
As one would intuitively suspect, we see that the return power drops as the square of the range. Likewise, return power also diminishes linearly as the illuminated area grows. Of course, the illuminated area grows quadratically with range as it is expanding in two dimensions once divergence of the laser’s beam commences. So, the signal return power drops as 1 ⁄Range or 1 ⁄Range depending on whether the target is before or after the commencement of beam divergence. It should be apparent that achieving long range requires emitting a lot of photons.
However, there are limits to the amount of laser power that can be used. Intense near-IR light (800 to 1400nm) can damage vision. As humans cannot see light in this range, we do not blink or avert our eyes to bright near-IR light. But our eyes can focus this light onto our retina. This can result in retinal damage. Longer wavelengths of light, 1400 to 3000nm (or short wave-IR) for example, are absorbed by the aqueous area behind the cornea . So while it is similarly invisible to humans, we can tolerate a lot more laser exposure at those wavelengths – roughly five orders or magnitude more. The reason this is important to understand with regards to direct detection LIDAR is that many LIDAR systems (particularly low-cost automotive LIDAR) use 905 or 940nm as their operating wavelength due to the wide availability of low cost InGaAs-based lasers and Silicon photodiodes. Lasers and photodiodes at short wave-IR tend to be much more expensive, negating the main advantage of direct detection – its simplicity and low cost.
There are other means to improve direct detection range by improving receiver sensitivity. Larger area receiving lenses can be used. Increasing the photon collection area offers improved receiver sensitivity without any additional electronic noise. Doubling the lens diameter offers 4x the receive sensitivity at the expense of a larger and more complex optical system (recall that a 16x increase in gain only translates to a doubling of range). A larger aperture transmit beam can be used to maintain tight collimation of the laser over a longer distance (see the section on Rayleigh range below), but large diameter beams may not be compatible with many scanning methods (small MEMS mirrors, for example). Avalanche photodiodes (APDs) – photodiodes with intrinsic gain – can be used to increase receive sensitivity. As a practical matter they can offer gains of about 5x to 15x before self-generated noise becomes a problem. Avalanche photodiodes tend to be expensive and fragile. They are also generally very small area devices, which complicates the optical design further. Finally, Geiger Mode Avalanche Photo-Detectors (GMAPDs) or Single Photon Avalanche Detectors (SPADs) are available. They offer extreme sensitivity – as little as a single photon is needed for detection. However, once they have been triggered, they require finite time (~5 to 10ηs), to recover before being able to trigger again . While these can make a highly simplified long range LIDAR system, their principle of operation is such that they are susceptible to interference (Solar and adjacent LIDAR systems) and work poorly in snowy, dusty, or foggy environments (a photon reflected off of a snowflake will blind the GMAPD to anything 1.5 to 3m behind the snowflake). As we will discuss later, some applications are not subject to interference from the Sun, adjacent LIDAR systems, or concerned about poor weather environments. In those applications GMAPD based direct detection systems work very well.
Regarding interference, it is also important to note that direct detection systems used in applications where there are other LIDAR systems around (like automotive or autonomous ground vehicles) must design-in some means of interference mitigation. To the receiver of a direct detection LIDAR system, every light pulse at a similar wavelength looks just like its own pulse. This is not a LIDAR exclusive problem. In the early days of automotive radar, pulsed systems were used. Once many cars were equipped with radar, mutual interference became a problem. In response, the automotive radar industry moved to coherent detection techniques – mostly FMCW – largely solving the mutual interference issues. In general, some kind of pulse coding must be used to distinguish “your” laser pulses from other systems. The cost to this is either reduced range (if average laser power is limited due to thermal or eye-safety issues) or a reduced number of spots/second the LIDAR unit is capable of measuring. Pulse coding is difficult to do when using GMAPDs as the time between pulses must be long enough to ensure the GMAPD has recovered from the last pulse.
Finally, it should be noted that direct detection LIDAR does not measure velocity (which can be a valuable input to downstream perception) directly . Velocity may be inferred by measuring target movement across multiple frames; however, this tends to be a low accuracy measurement technique as it depends on repeatable measurements of the target position in each frame. For example, if a target is moving at 15m/s (about 33mph) and frame rate is 20Hz the target would have moved 75cm in one frame. If measurement accuracy is ±10cm (about the best one would expect from a direct detection automotive LIDAR system), then velocity measurement error could be as high as ± 10cm/75cm = ±13%. Of course, this could be improved by measuring multiple consecutive frames. But this would take time as measurement accuracy only improves with the square root of the number of measurements taken (for example, 9 averaged measurements improve the accuracy by a factor of 3 while increasing latency by a factor of 9, up to 450ms at the frame rate of 20Hz).
To continue reading the full whitepaper for free, click HERE.
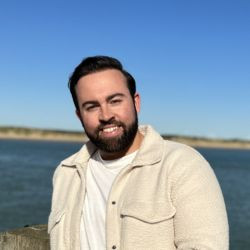